|
Archives
and Download - Archives
Three-Colour,
Solid-State, Three-Dimensional Display
E. Downing, Mechanical Eng. Dept., Stanford
University, Stanford, CA 94305-4035, USA;(415) 725-3288, downing@kaos.Stanford.edu.
L. Hesselink, Electrical Eng. Dept., Stanford University, Stanford, CA
94305-4035, USA.
J. Ralston, SDL Corp., 90 Rose Orchard Way, San Jose, CA 95134-1365, USA.
R. Macfarlane, IBM Almaden Research Center, 650 Harry Road, San Jose, CA
95120, USA.
Abstract
A three-colour, solid-state, volumetric display based on
two-step, two-frequency upconversion in rare earth doped heavy metal
fluoride glass is described. The device employs infrared laser beams
that intersect inside a transparent volume of active optical
material to address red, green, and blue voxels via sequential
two-step resonant absorption. Three-dimensional wire-frame images,
surface areas, and solids are drawn by scanning the point of
intersection of the lasers around inside the material. The prototype
device is driven with laser diodes, uses conventional focusing
optics and mechanical scanners, and is bright enough to be seen in
ambient room lighting conditions.
|
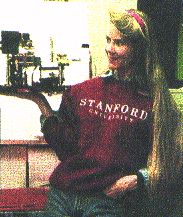
Dr. Elizabeth Downing |
Introduction
The ability to visualise real-time volumetric data in true
three-dimensional form has been a goal of display development efforts for
many years. Several clever techniques have been developed, but none has
provided a complete solution for the need to view dynamic volumetric data in
a realistic interactive format. In this paper we present a volumetric
display technology that is capable of displaying real-time three-dimensional
objects in true 3D spatial form. The device can be viewed from any
perspective, through all sides of the display volume, and does not require
the assistance of any special glasses or headgear. The device features
unencumbered viewing access to multiple users simultaneously, and is capable
of displaying information using the three additive primary colours red,
green, and blue.
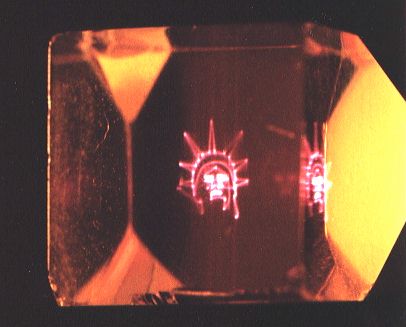
3D image scanned by IR lasers
in a glass cube
Background
Three-dimensional perception is a complex cognitive process involving the
eyes and the brain as a visual system. Historical solutions to displaying 3D
information are diverse and varied, and have often relied on tricking the
human visual system into thinking that it is seeing a 2D scene in three
dimensions. While specific system requirements vary for different
applications, a successful three-dimensional display should provide the
viewer, to the necessary extent, with a number of intrinsic visualisation
parameters. These parameters include the field of view, which is defined by
the solid angle subtended by the object, and thus determines the apparent
size of an object as seen by the viewer, and the viewing zone, which is
defined as the range over which the user or users can move and still see the
objects displayed clearly in the field of view.
Autostereoscopic viewing is the ability for the displayed objects to be
continuously viewable from all regions within the viewing zone, and
accommodation is the ability for the user to focus on selected depth planes
within the object. Dynamic and real time capabilities allow moving objects
to be displayed at a refresh rate sufficient to avoid flicker (30-60 Hz),
and interactive capability allows the user to modify objects being
displayed, i. e., zoom, translate, rotate, cut and paste. Colour refers to
the ability to address three primaries such as the additive red, green, and
blue (RGB), from which to produce a wide spectrum of colours, including
white, by appropriate mixing.
Many of the systems that have been developed to date, such as holographic
and stereoscopic displays have limited viewing zones and usually present
static images, or images with very low resolution. Others, like virtual
reality displays require the user to wear special headgear that interferes
with normal visual processes. Display approaches in which two-dimensional
reflective or emissive surfaces are swept throughout a third dimension have
been demonstrated, but they too have restricted viewing zones and require
large surfaces to be deflected or rotated at high speeds.
The traditional two-dimensional cathode-ray tube (CRT) and liquid crystal
displays (LCD), in which dynamic 3D scenes can be rendered using appropriate
graphic constructs such as shading, shadowing, and perspective, have thus
far received the highest degree of implementation for visualising many types
of three-dimensional data. These systems however, display 3D information
from only one viewer perspective which prevents the user from employing
natural human depth cues such as stereo vision and motion parallax.
Multiplexing methods that have attempted to introduce stereoscopic viewing
capabilities into CRT displays have resulted in reduced viewing zones, and
the need for the user to wear glasses or tracking headgear. None of the
approaches that have been presented thus far has provided a complete method
for displaying dynamic volumetric data without intrinsic limitations to
visualisation.
The display concept described in this paper has been demonstrated in a small
scale proof-of-principle prototype, and provides a solution in which all of
these visualisation parameters have an attractive range of values. A
previous attempt to demonstrate this technique, made by Lewis, et. al., of
Battelle Laboratories in the early 1970s succeeded in generating two faint
spots of light inside a crystal of erbium doped calcium fluoride using
filtered xenon lamps as excitation sources. The pioneering work of this
group of researchers pointed out the potential advantages of such a display,
and indicated that the shortcomings were primarily associated with the lack
of suitable excitation sources and the lack of materials with sufficient
infrared to visible conversion efficiency.
We have utilised high power laser diodes in our work and improved
upconversion materials, to lay the critical foundation for this 3D display
technology. Of particular importance to the technique are the absorption
cross sections and intermediate level lifetimes of the active ions, and the
spectral distribution of the fluorescence. In this paper we describe the
fabrication and optimisation of upconversion materials at Stanford
University, and the integration of these materials with laser diodes and
scanning systems to create a three-colour, solid-state three-dimensional
display.
Principle of
Operation
The physical mechanism upon which this 3D display
technology is based is known as two-step, two-frequency (TSTF)
upconversion. This phenomenon occurs when an active ion, which has
been doped in small quantities into a bulk transparent host
material, is optically excited to higher energy levels by absorbing
energy from two different-wavelength, near infrared (IR) laser
beams. The active ion, which normally occupies its lowest energy
level E0, can absorb energy from the first IR laser beam l1, making
a transition to the intermediate excited state E1, where it will
stay for the lifetime t1, of this level. If the second IR laser beam
impinges on this ion while it is in the first excited state, it will
absorb energy at the second laser wavelength l2, and undergo a
transition to the second excited state E2. An ion that has been
excited in this manner to the higher level E2, has effectively
summed the energy it absorbed from both pump photons, and can
re-emit most of it as a single photon of visible light by decaying
back to the ground state. |
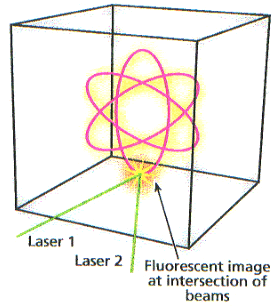 |
It is crucial that the two-step excitation process in the active ion
occur only from the selective absorption of two different infrared
wavelengths, as it is this mechanism that enables a visible point of light
to be "turned on" only where the two laser beams cross, and
nowhere else. By controlling the spatial co-ordinates of the intersection of
the two lasers, a "voxel", or volumetric pixel, can be addressed
at a specific location inside the bulk imaging medium. Rapidly scanning the
point of intersection around inside the display volume, moves the position
of the voxel, and allows three dimensional images to be drawn.
Description of
Prototype Device
The photograph above simple images that have been drawn in our prototype
solid-state 3D display. The photograph was taken in ambient room lighting
conditions and all the objects drawn in the display are easily visible. Red,
green, and blue Lissajous figures were created in a stack of three
individual glass layers that were laminated together with index matching
optical adhesive to form a composite structure. Each layer was doped with a
different ion and was pumped with the wavelength combination appropriate for
that dopant.
All of the wavelengths required to resonantly pump each of the necessary
dipole transitions in these rare earth ions are commercially available as
laser diodes at power levels greater than 100 mW from SDL Inc. (Table 1).
Simple mechanical scanners, driven by function generators at rates of 30 Hz
to 100 Hz were used to deflect the laser beams into the 1.5 cm x 1.5 cm x
1.5 cm composite sample. Both resolution and voxel size in this display are
determined by the diameters of the intersecting laser beams which when
focused to 100 mm spot sizes, would produce roughly 300 voxels along the
perimeter of a 1.0 cm diameter circle.
The transparent host materials used for this display are heavy metal
fluoride glasses (HMFG) doped with praseodymium (red), erbium (green) and
thulium (blue) rare earth lanthanides . These glasses, the most common of
which is ZBLAN have been developed for fiber laser and optical amplifier
applications and are characterised by low (<500 cm-1) phonon energies, a
critical parameter leading to reduced nonradiative losses and increased
upconversion efficiencies. Low-phonon energy glasses offer several distinct
advantages over crystalline material hosts. They are relatively easy to
manufacture enabling device performance parameters to be investigated in a
wide range of compositions. In addition, they can be easily cast into a
variety of shapes from which to construct a display volume.
Glasses are isotropic and have no polarisation restrictions on pump lasers,
assuring that light is emitted isotropically from any voxel. Glasses also
introduce inhomogeneous broadening into the electronic transitions of the
active ions, creating absorption resonances that are several tens of
nanometers wide. While this feature facilitates pumping with laser diodes,
as absorption is insensitive to wavelength drift, feedback susceptibility,
and astigmatic focusing, it can reduce the absorption cross sections and
increase single frequency upconversion. Glass ceramics , which offer the
fabrication ease of glasses along with increased absorption cross sections,
may provide improved materials for this display technology.
Various fluoride glasses were fabricated by melting optical grade
precursor materials in vitreous carbon crucibles under a reactive atmosphere
of 3% Cl2 (balance N2). Melting temperatures between 700o C and 850o C were
used, with homogenizing soak cycles ranging from 1.0 to 1.5 hours. Rapid
quenching of the molten sample material was accomplished by removing the
crucibles from the heat source and introducing connective cooling with dry
N2 gas. Samples on the order of tens of cm3 with no observable crystals
under 20X magnification were routinely prepared.
Detailed Description
of Upconversion Processes
The trivalent rare earths are well known for their ability to absorb
infrared energy and become excited to higher metastable quantum states.
Their 4f electronic levels are well shielded from the ligand fields in
crystalline and vitreous hosts by the outer 5s and 5p electrons, yielding
long lived metastable lifetimes. Figure 3A show the energy level diagrams of
the lanthanide ions used in our display, along with the pump laser
wavelengths and fluorescent emission lines. The 2S+1LJ notation used to
label the various levels in these ions refers to the spin (S), orbital (L),
and total (J) angular momentum quantum numbers of the free ions. Selection
rules based on these quantum numbers govern transition probabilities between
the atomic levels.
A steady state rate equation analysis of two-step, two-frequency excitation,
using a simplified 5-level model, shows how the total power Pv, emitted from
a single voxel at the intersection of the two resonant pump laser beams,
depends on the various excitation and material parameters: Pv = x ()
NT V s1 s2 t1. (1)
In equation 1, P1 and P2 are the powers of the two pump laser beams and A is
the area of the focused spot, is Planck's constant, wij are the
transition frequencies between the levels, NT is the dopant concentration, V
is the voxel volume, s1 and s2 are the ground state and excited state
absorption cross sections, and t1 is the lifetime of the intermediate level.
The term x is the fraction of the light emitted in the useful bandwidth, and
can be obtained from a calculation of the branching ratios to the different
final states.
Equation 1 assumes perfect overlap of the two pump lasers. Using a 0.5% (NT
= 2.5x1019 cm-3) erbium doped HMFG, which has an intermediate lifetime of 15
ms, and ground state and excited state cross sections on the order of 10-20
cm2, a 100 um3 voxel can be excited to emit 0.5 uW of total power (the
equivalent of that emitted by a single pixel in a high intensity CRT or LCD
display) with approximately 20 mW of pump power from each laser. This
assumes optimum pumping conditions in which the wavelengths of both lasers
are resonantly matched to the transition frequencies wij, of the dopant.
Voxel brightness is maximised when the excitation periods are greater than
or equal to the intermediate level lifetime t1. The lifetime of the
intermediate E1 level, should be long enough to allow efficient filling by a
cw laser, but short enough to prevent "ghost" voxels from being
created at previously scanned locations. The lifetime of the excited E2
level, should be short enough to allow dynamic objects to be drawn without
smearing the image.
Commercial diode lasers are capable of output powers from hundreds of
milliwatts to several watts (7 ) and can readily provide the pump power
needed to implement this 3D display technology. Materials with larger
absorption cross sections and longer intermediate lifetimes will provide
better performance by reducing the power requirements of the display.
Red upconversion fluorescence was induced in trivalent praseodymium, Pr3+,
doped fluoride glass by pumping with 1014 nm and 840 nm respectively. The
absorption cross section of the 3H4 to 1G4 transition is small, partly
because it is forbidden by spin selection rules. The 1G4 level has a
lifetime as long as 0.325 ms depending on the material host and dopant
concentration . The 1G4 to 1I6 transition in the Pr3+ ion has an absorption
cross section that is two orders of magnitude higher than the ground state
cross section (14). Energy absorbed into the 1I6 level, quickly relaxes to
the nearby lower 3P0 level via nonradiative processes, and is emitted
predominantly as red light at 605 nm (3P0-3H6) and 636 nm (3P0-3F2).
Two-step, two-frequency upconversion fluorescence to green was induced in
trivalent erbium doped fluoride glass. All of the relevant transitions in
erbium are spin allowed yielding high absorption cross sections. The green
emission shown is at 545 nm and has been induced in an erbium doped sample
using pump wavelengths of 1.5 mm and 850 nm. It is important to note that
erbium doped materials are also subject to single frequency upconversion
when pumped with 1.5 mm, especially at dopant concentrations greater than a
few mole percent. This phenomenon has been used to make single frequency
pumped erbium upconversion lasers but is detrimental to the TSTF absorption
processes needed for 3D display applications, as it will make the 1.5 mm
pump laser beam itself visible inside the display volume as a green line.
Single frequency upconversion in erbium is a higher order, intensity
dependent process that requires at least three 1.5 mm pump photons to be
absorbed to produce one at 545 nm. Scanning the pump laser beams inside the
display at a sufficiently high rate decreases the excitation period, and
reduces single frequency upconversion in erbium to a magnitude where it is
not noticeable to the viewer.
Upconversion fluorescence to blue at 480 nm, was induced in trivalent
thulium doped fluoride glass . The first transition in thulium (3H6 - 3H4)
is resonant with 800 nm, and the second step with 1120 nm. The square, blue
surface area shown in the photograph was pumped with a second step
wavelength of 1064 nm from a Nd:YAG laser. Although the second pump
wavelength was more than 60 nm off resonance from the peak absorption
frequency of the transition, the TSTF process was still induced in the glass
host. While this illustrates the advantage of using vitreous materials when
pumping nonresonantly, exactly resonant excitation of this transition will
lower the power requirement.
Figure 4 shows the spectral content of the red, green, and blue fluorescence
obtained from the TSTF pumping schemes in praseodymium, erbium, and thulium
doped fluoride glasses. While each of these ions also yields some emission
at other wavelengths, the primary colour components are in the indicated RGB
bands, and it is merely fortuitous that the spectral peaks provide three
saturated primaries encompassing the human visual colour gamut.
Conceptual System
Architecture
A variety of system architectures can be envisioned in which to implement
this solid-state 3D display concept to provide multiple, individually
addressable colours. The three colours were drawn in materials with dopant
concentrations of around 0.5 mole percent, a value that has been
experimentally determined to maximise voxel brightness. Higher
concentrations of the dopants lead to decreased upconversion efficiency due
to cross relaxation between the ions which depletes the populations of the
intermediate levels. This, unfortunately, has prevented codoping all three
ions into a single monolithic bulk medium to obtain wavelength addressable
colour. The problem of mixing the dopants is compounded by the pump
wavelengths used to induce TSTF upconversion in one ion, inducing single
frequency upconversion in another ion, even at total dopant concentrations
below 0.1 mole percent. Single frequency upconversion in other than the
target ion causes the infrared pump wavelengths to show up as visible lines
in the display, and causes the spectral content of a single voxel to be
composed of emission lines from multiple dopant ions with an overall voxel
brightness that is weaker in magnitude.
A better way to achieve a large scale three-colour system may be to
implement a composite structure consisting of a repeated sequence of three
singly-doped layers. In this configuration, the three individual colours
would be addressed in three different thin (100 mm - 500 mm) material
layers, each of which has been optimised with a different dopant and host
glass composition. A broad colour spectrum, including white, could be
created by controlling the intensity ratio of voxels in three adjacent
layers, in much the same way as colour is created by addressing three
closely spaced pixels in a CRT display.
Layering the display volume provides the added feature of spatially
separating the wavelength pairs required to address each colour. This is an
important consideration as erbium, for example, will undergo
single-frequency upconversion when pumped with the excitation wavelengths
for thulium and praseodymium. A three-layer stack of individual colours
could be repeated over and over again to form the total display volume. This
concept offers complete wavelength addressable RGB capability with one third
the resolution of a monochrome device.
A layered material system also intrinsically incorporates a
parallel system architecture into its design. We define hp, the single voxel
pump efficiency of the material, as the ratio of the total power emitted
from a single voxel (Pv) divided by the total power (PT = P1 + P2) of the
two input pump lasers, (hp = Pv/PT). Simple calculations show that the total
power required to pump N3 voxels can be given by PT = N3 Pv 1/hp, or ET /tex
= N3 Ev /tex 1/hp, eq. 2 - where ET is the pump energy, Ev is the energy
emitted by the voxel, and tex is the excitation period. Equation 2 shows
that a voxel need not emit light continuously, but can be excited to emit a
higher amount of energy for a shorter period of time (Pv = Ev/tex), if it is
pumped strongly and refreshed again a short time later. A single voxel (N=1)
that is pumped with 30 times the excitation energy for 1/30th of a second
(PT = ET/tex), and refreshed once a second, will emit the same average power
as one that is pumped continuously at PT. In order to address N3 voxels
every 1/30th of a second using only two lasers, the scan rate must be N3*30
Hz. A 3 MHz scan rate would be needed to address 100,000 voxels, producing
an excitation period of 0.3 ms per voxel (RS = 1/tex), and requiring a pump
energy 100,000 times greater than that necessary to turn on a single voxel
at 30 Hz.
A pump energy this high would be above the damage threshold of most
optical materials. The inadequacy of using only two pump lasers arises
because only a small portion of the available power along each beam (~ 100
um) can be utilised at any particular instant to excite a voxel, leaving the
remaining length of each beam to go unused. The overall efficiency of this
system can therefore never be greater than the single voxel pump efficiency
of the material, hp. If, on the other hand, a system design using parallel
pump lasers to simultaneously excite multiple voxels along the length of
each beam is employed, more of the available power in the lasers could be
utilised, reducing scan speeds and data rates, and lowering the power
requirements of the system. For example, two orthoganally-positioned linear
laser diode arrays with one hundred emitters each, can address 10,000 voxels
in a plane when turned on simultaneously.
This yields a device efficiency equal to 100 times hp (100 P1 + 100 P2 =
10,000 Pv), or two orders of magnitude greater than a two laser system
pumping 10,000 voxels. Lowering the power of the lasers is also attractive
from a safety standpoint as any pump power that is not absorbed by the
active medium will require filtering at the viewing surface of the display.
Parallel system architecture, combined with appropriate multiplexing
algorithms, would increase the device efficiency of the display beyond the
single voxel pump efficiency hp of the material, by allowing for more
effective utilisation of available pump power.
Monochrome systems in which only one colour need be addressed would require
only one dopant ion in the display medium and could be implemented with a
similar parallel excitation architecture. In this case, the display volume
could be either monolithic, or constructed compositely from layers or blocks
of material which could be substantially thicker than the layers needed for
a colour display. These layers could vary in dopant concentration, with
higher doped layers placed farther away from the laser pump sources,
producing an absorption gradient.
This design could be used to balance the reduction in pump power that occurs
as the ground state laser beam propagates through the material and is
absorbed. A layered structure would also help to reduce some of the
practical issues associated with casting a large monolithic piece of low
phonon energy glass, as these materials have a low thermal conductivity and
are difficult to fabricate in pieces thicker than a few inches. This would
enable large displays having several inches on a side to be manufactured.
Conclusion
The solid-state 3D display technology described in this paper can be
driven entirely with commercially available laser diodes and scanning
systems, and can be powered directly from 120 V, 60 Hz electrical outlets.
The display operates at room temperature, and is viewable under typical
ambient room lighting conditions. Current efforts are under way to implement
a more fully integrated system architecture, and to increase upconversion
efficiencies with improved material systems.
We believe that this technique offers a viable approach for presenting
real-time multi-dimensional information to a multitude of viewers,
independent of viewing perspective, with no obstructed viewing regions, and
no need for special viewing eyewear.
The authors would like to acknowledge Randy Klein and Dave
Evans of SDL Corp. for their valued technical assistance with laser diodes,
and Nick Borrelli and Roger Bartholomew of Corning Inc. for performing
lifetime measurements. Elizabeth Downing would like to personally thank John
Trias and Parvis Soltan of NRaD, two visionaries who believed in her enough
to fund this research at Stanford three years ago under contract
#N6600-92-D-0092. This work was also supported in part by L. N. Durvasula of
ARPA under contract #N0014-92-J-1903 through the Stanford Center for
Nonlinear Optical Materials (CNOM).
DISCLAIMER:
Some of the information in the Backstage area is provided by the persons or
companies named on the relevant page(s). Laser F/X does NOT endorse or
recommend any products/services and is NOT responsible for the technical
accuracy of the information provided. We provide this information as a
service to laserists using the Backstage area.
[ Introduction
| Archives | Download
]
|